Narrative Review
An Avant-garde Approach to Life: Reviewing the Current Applications of 3D Bioprinting
Iqra Nawaz1, Yashfa Nawaz2, Eisha Nawaz1
Published online: June 23, 2022
1Quaid-e-Azam Medical College, Bahawalpur, Pakistan
2Army Medical College, Rawalpindi, Pakistan
Corresponding Author: Iqra Nawaz, email: iqranawazabbasi@gmail.com
DOI: 10.26443/mjm.v20i2.919
Abstract
Introduction: The promise of bioprinting tissue constructs that could potentially serve the same function in the human body as native tissues has taken the world of regenerative medicine by storm. The current review describes system-wide clinical applications of three-dimensional (3D) bioprinting and aims to address ethical and social considerations, while also discussing the scope of this technology in the near future.
Discussion: 3D bioprinting is believed to present new approaches to conventional treatment; offering the advantage of customization and on-time availability. It facilitates simultaneous deposition of appropriate bioinks and biomaterials onto scaffolds which can then be employed to develop tissue fabricates that can potentially mimic native tissues in both structure and functionality. It has been extensively employed to regenerate viable tissue constructs of skin, bone, cartilage, vasculature, myocardial tissue and heart valves, nervous tissue, lung and tracheal tissue, liver, pancreatic, and corneal tissue.
Conclusion: To obviate the current restrictions associated with this technology, it is imperative to understand where we currently stand in terms of current clinical applications of 3D bioprinting. This technology is anticipated to contribute significantly to the fields of tissue engineering and regenerative medicine (TERM), where it can be employed to fabricate functional tissues that can simulate their counterparts in the human body.
Relevance: The increasing disparity between organ demand and supply as well as the shortcomings associated with antiquated approaches to treatment call for utilizing 3D bioprinting to develop viable tissue constructs.
Tags: Bioprinting, Tissue Scaffolds, Biomaterials
Introduction
Organ transplantation is the ultimate approach for the treatment of end-stage diseases. A precarious imbalance between organ demand and organ supply accentuates the need for bioprinting viable tissue fabricates that can simulate the physiological and anatomical features of target tissues and can serve the same function in vivo as their counterparts in the human body. 3D bioprinting is defined as the process of depositing biocompatible materials in a layer-by-layer manner to develop tissues that can mimic the properties of living cells (1). The creation of tissue constructs is performed by combining computer assisted design (CAD) with computer assisted manufacturing (2) to meticulously fashion appropriate biomaterials and bioinks into tissue substitutes; and provides considerable control over their structure, reproducibility, and functional accuracy (3). This technology offers concurrent printing of various types of cells in defined spatial locations, making its use in regenerative medicine of paramount importance (4). This review aims to highlight current applications of 3D bioprinting in clinical settings as well as pharmaceutical and cancer research; and, moreover, will recount socio-ethical concerns, future perspectives and possibilities.
Approaches to 3D Bioprinting
Techniques used to bioprint tissue constructs are grouped into various categories based on their printing principles. These categories include inkjet-based, pressure-assisted, laser-assisted bioprinting techniques, stereolithography, and extrusion based bioprinting (5); and each technique finds its application in printing specific tissues, having particular advantages and shortcomings (6). Salient features of commonly employed approaches to bioprinting have been summarized in Table 1. Bioinks, which are a crucial component, refer to cellular aggregates deposited on or within scaffolds or a construction of cells that may consist of bioactive components and biomaterials (7). Clinical applications of 3D bioprinting have largely been restricted by the lack of adequate bioinks available for printing viable target tissues (8) and limitations associated with bioprinting techniques, including poor resolution and deformation of cells due to shear stress (9).
Table 1: A summary of salient features of major bioprinting techniques along with their clinical applications. | 3D Bioprinting Technique | Process | Advantages | Limitations | Applications |
Inkjet-based | Droplet-On-Demand technique. Involves one of the techniques mentioned below (6) to dispense droplets onto a collection platform to allow build up of layers in the z direction and eventual construction of a 3D structure (5). | Contactless printing. Allows for modulation of size, position, and deposition rate of droplets. High spatial resolution (38). High cell viability (80-90%) (5). | Cannot use high viscosity materials. Mainly used for printing small scaffolds (38). | in situ bioprinting of skin (60) Blood vessels (35) Human dermal fibroblast (5) Bone and cartilage constructs (5) |
Peizo-assisted | Glia and retinal ganglion cells (36). | |||
Thermal-assisted | Cellular viability is affected by heat application (36). Nozzle clogging (36). | |||
Pressure-assisted | Involves the use of plastic or glass cartridge filled with selected bioinks/biomaterials, ejected in the form of a filament through a nozzle or a needle (36). | Allows dispensing of different combinations of cells and biomaterials (36). | Low resolution. Improving resolution requires decreasing nozzle diameter which results and low cell viability (36). | Cells such as murine pre-osteoblasts, human mesenchymal stem cells, endothelial cells, and osteogenic progenitors (6). |
Laser-assisted | Involves a tri-layered system of an energy-absorbing layer, a donor layer and a bioink layer (5). Application of a laser beam to desired sites of energy absorbing layer results in vaporization of corresponding locations of donor layer underneath, leading to the formation of a high-pressure bubble at the interface. This results in impelling of bioink, leading to a droplet falling onto the platform (5). | Nozzle free and so no clogging. Can utilise bioinks of relatively higher viscosity. Allows manipulation of single cells. Allows precise control of position of cell droplets. Fast bioprinting speed (38). | Although resolution is very high, it is dependant on multiple factors such as viscosity of bioinks, speed of laser printing, etc. Laser-induced cellular death during the process leads to low ultimate viability (38). | Human dermal fibroblasts, Graftskin skin substitutes (6). Cornea (49) Bovine pulmonary artery endothelial cells, breast cancer cells, mural neural stem cells (6). |
Stereolithography | Utilises photo-polymerisation to solidify bioinks in a layer-by-layer fashion along the z-axis, resulting in formation of complex 3D structures from 2D pattern of interest (5). | First used in reconstructive head surgery to produce accurate models of the cranium (5). Relatively higher bioprinting speed (5). No shear stress is applied to the cells (5). High cellular viability (5). High resolution (5). High Fabrication Accuracy (6). | The liquid used must be transparent with minimum scattering of light (5). Only certain densities of bioinks can be used (5). Unavailability of biocompatible and biodegradable polymers. An inability to completely remove the supporting structure and to form horizontal gradient in the constructs (6). | Alveoli (31) Bone tissue (35) |
Extrusion bioprinting | Continuous extrusion of bioink from the nozzle tip (38) driven by three types of systems: pneumatic, piston and screw. The pneumatic system dispenses bioink using compressed gases, piston and screw systems use mechanical forces without gases, dispensing bioink through a pump (13). | High deposition and printing speed (9). Allows bioprinting of a wide range of bioinks with high cellular density, and of anatomically correct porous constructs (9). Printed scaffolds show good structural integrity (38). Fast printing speed (14). Can produce large cell-laden constructs in a controllable manner (14). | Biomaterials need to have high elastic modulus and an appropriate loss modulus. The consistency of the biomaterial should be fluid-like so it can be extruded through the nozzle. Extrusions must be strong so that shape can be maintained, both during and after the process. Relatively low printing resolution. Stress-induced cellular distortion. Low cellular viability (38). | Bone (19) Cartilage (21) Cardiac tissue (20) Nervous tissue (microextrusion bioprinting) (29) Blood vessels (35) Liver (43) Pancreas (47) Cornea (48) Skeletal muscle (35) Hepatocytes (9) Lung tissue analogues (9) Renal organoids (39) |
Current Applications
3D Bioprinting has surfaced as a major scientific breakthrough in the rapidly advancing fields of regenerative medicine and medical research (10). Over the last two decades, it has challenged the obsolescence of conventional treatment, offering an effective solution to problems such as insufficient matching organ donors, post-transplant immune rejection, and infection. Current major applications of 3D bioprinting have been summarized in Figure 1.
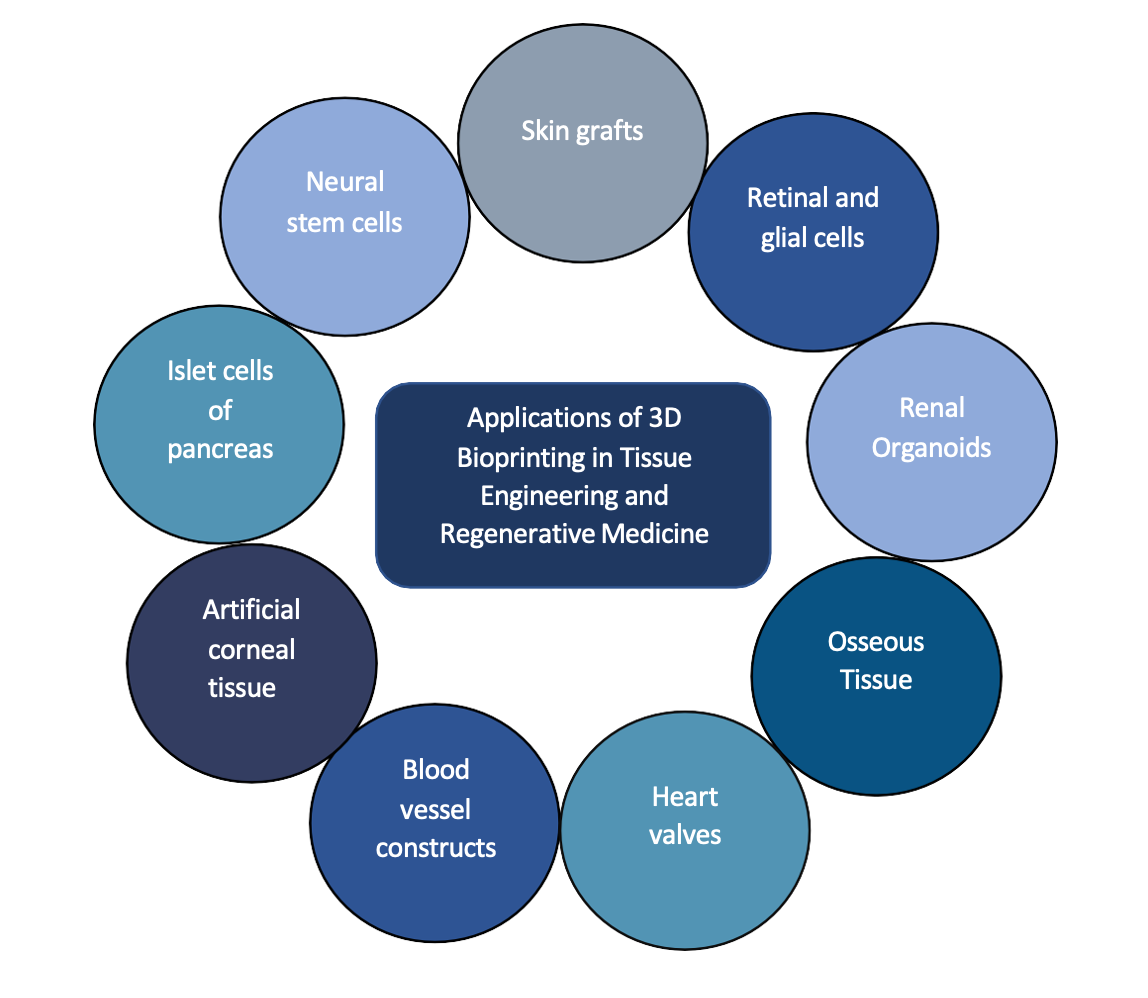
In Tissue Engineering and Regenerative Medicine
The organ demand/supply disparity can be curtailed by cell and tissue fabrication now made possible through 3D bioprinting; and has been extensively used to regenerate skin, bone, cartilage, myocardial tissue and heart valves, lung and tracheal tissue, liver, blood vessels, pancreas, and corneal tissue.
Skin
Skin, being the outermost layer, is extremely susceptible to damage resulting from disease, trauma, burns, or surgery, making skin restoration imperative post-trauma or injury. The bioink employed in skin engineering is an optimal combination of cells, typically keratinocytes and fibroblasts collected from the patient, and collagen, which is used as hydrogel to imitate the extracellular matrix (ECM) (11). This combination is then printed using the appropriate bioprinting technique following digital photographs or thermal images (11). Skin bioprinting becomes particularly important in the treatment of full-thickness burns where, in the place of severe burn dressings, multilayered skin substitutes are being developed using cells and ECM combined as bioinks to counter the expense of treatment and scarcity of donors (12). Although skin bioengineering offers a promising solution to the shortcomings of autotransplantation, allotransplantation, and xenotransplantation, which include transplant rejection, risk of infection, and limitation to minor injuries (13), the clinical application of skin constructs is largely limited by the challenge of how to vascularize and innervate said constructs (14).
Bone
Although known to have highly regenerative and reparative properties, osseous tissue often fails to heal large-scale bone trauma completely by itself (15), whereby clinical intervention becomes necessary to ensure normal bone function; and 3D bioprinting of bone tissue offers numerous advantages pertaining to control over porosity, biocompatibility, mechanical strength, and degradability (15). Bone tissue is comprised of osteoblasts and inorganic ECM, where inorganic ECM is composed primarily of hydroxyapatite (HA) (16). HA and tricalcium phosphate ceramics are commonly employed as bone scaffolds, offering a favorable environment for cells like osteoblasts and mesenchymal stem cells (MSCs) to grow (17). Importantly, constructs synthesized using scaffolds of HA and polycaprolactone (PCL) that are implanted into skull defects exhibit vascularization, integration with surrounding tissue, and provide support for new bone formation (18). Currently, such bone graft substitutes are predominately cell-free and rely on host progenitor cells to initiate the process of bone recuperation (18). Constant enhancements to bone constructs are being made by the addition of effective growth factors, investigation of better biomaterials, and improvement of bioprinters to ensure not only implantation of these constructs but also their sustainability over time. Of great importance when considering enhancements to bone constructs is successful vascularization, which is crucial for the supply of nutrients and has been achieved via in vivo strategies that utilize smart scaffolds and pre-vascularized grafts (19).
Cartilage
Commonly occurring degenerative cartilage diseases, such as osteoarthritis, along with the limited regenerative potential of articular cartilage call for cartilage bioprinting to combat the restrictions associated with current treatment options. Because cartilage is an avascular tissue, this makes it one of the simplest structures to be successfully bioprinted. Similar to the anatomical and physiological properties of chondral tissue, 3D bioprinting is able to deposit chondroprogenitor cells or differentiated chondrocytes along with suitable growth factors in biocompatible scaffolds to attain the functionality of native tissues (20). Although chondrocytes are predominantly used for cartilage bioprinting (21), the use of stem cells instead offer a better opportunity to improve cartilage regeneration due to their immune privileged status and paracrine activity (22). For the bioprinting of cartilage, bioinks utilized for the synthesis of hyaline cartilage are most commonly alginate and agarose hydrogels seeded with MSCs, and GelMA (gelatin methacrylate) for the synthesis of fibrocartilage (23).
Cardiac Tissue
Significant research efforts in 3D bioprinting have focused on ways to bioprint cardiac tissue in hopes of better treating cardiovascular disease, which is the primary cause of death worldwide (24). As far as bioprinting of cardiac tissue is concerned, conservation of mechanical and electrical properties of cardiomyocytes remains a key requirement (25). Tissue spheroids have been constructed by co-printing human vascular endothelial cells (HUVECS) and cardiac cells which, when fused together, establish a cardiac patch after approximately three days that is able to beat in sinus rhythm (20). Importantly, contractility and conductivity of cardiac constructs are reported to be improved by applying electrical stimulation during the culture of these constructs (26). Despite prosthetic valves previously used in treating valvular heart disease being successfully substituted by 3D printed heart valve conduits (synthesized using methacrylated hyaluronic acid and methacrylated gelatin) (27), bioprinted cardiac tissue, as of now, is far from implantation-ready at present stage. (20)
Nervous Tissue
The central nervous system represents considerable intricacy of structure, immense complexity of function as well as a weak capacity for regeneration and, therefore, poses a major challenge for the synthesis of viable neural tissue constructs. Impressively, human cortical spheroids (hCSs) containing neurons from deep and superficial cortical layers have been developed from pluripotent stem cells, and, moreover, are able to exhibit spontaneous activity, form synapses, and recruit networks of astrocytes (28). Alternatively, a polysaccharide-based bioink comprising of alginate, carboxymethyl-chitosan and agarose used in conjunction with direct-write printing of human neural stem cells (NSCs) facilitates in situ differentiation into functional neurons and supporting neuroglia (29). More recently, a poly(3,4 ethylenedioxythiophene)/chitosan/gelatin (PEDOT/Cs/Gel) scaffold used for 3D culture of NSCs has been reported to not only promote the adhesion and proliferation of NSCs but also facilitates their differentiation into neurons and astrocytes (30). Since only a few studies have been reported in the context of bioprinting nervous tissue, rigorous efforts are needed to further establish stable and functional nervous tissue constructs. Neural bioprinting, if and when applied clinically, may potentially solve the colossal challenge of innervation of bioprinted tissues.
Lung and Tracheal Tissue
3D bioprinting offers an advanced platform for concurrent deposition of multiple cell types in an accurate morphology so that the prerequisites for constructing lung-like tissues can be realized and appropriate scaffolds can be designed. Greater progress has been made in bioprinting tracheal tissue, however, epithelialization and vascularization of grafts remain as primary concerns (31). Even greater obstacles remain with regards to 3D printed lung tissue, as scaffolds should allow for proper gas exchange and account for other mechanical properties such as lung compliance, elasticity and recoil. To tackle these challenges, bioprinting is being utilized to develop a human air-blood tissue barrier analogue composed of alveolar epithelial type II cells, endothelial cells, and BM MatrigelTM via a valve-based bioprinting approach (32). Making this endeavour feasible is acellular collagen, MatrigelTM and alginate, which are commonly utilized as bioinks for the 3D printing of lung tissue due to their favorable elastic moduli (33).
Blood Vessels and Cellular Components of Blood
Printing biomimetic blood vessels remains a formidable challenge as a high resolution is required to print these extremely fine structures that must endure high stress and pressure without damage to the integrity and stability of the printed cells. Bioprinting blood vessels can be achieved by (a) the development of bulk matrices with integrated channels as perfusable matrices, (b) cell patterning into line structures for self-assembly of an interconnected vessel system, or (c) generation of free-standing tubular structures serving as artificial vessels (34). The addition of magnetically controlled nanoparticles (NPs) to bioink has been reported to keep the diameter of the blood vessel reduced to that of a capillary, yet, the use of magnetic NPs and magnetic fields in the positioning of vessel constructs within tissue is still in its infancy and requires greater investigation (35). Because a limited number of techniques are available to vascularize bioprinted tissue constructs, this hinders their implantation in vivo as adequate blood supply is needed for proper supply of nutrients and waste removal. So far, spheroid bioprinting upon appropriate biomaterial beds or direct deposition of cells on hydrogel scaffolds are two approaches employed to construct vascular structures. The former technique, however, results in constructs with low mechanical strength and stability (36) and, more generally, considerably more research is needed to generate functional and viable blood vessel constructs that can stand on their own. As far as cellular components of blood are concerned, a novel soluble nanobiotechnological complex formed by crosslinking hemoglobin, superoxide dismutase, catalase and carbonic anhydrase can serve all three functions of red blood cells. These include oxygen-carrying and carbon dioxide-carrying properties along with the ability to remove oxygen radicals, thus enabling successful preparation of artificial red blood cells (37).
Renal Tissue
Kidney damage primarily due to renal disease or secondary to Diabetes Mellitus, hypertension, or obesity that results in impaired kidney function is a grave health concern worldwide. Due to its intricate and complex structural morphology, only specific portions of the nephron have been synthesized, which are indeed similar in anatomy and physiology to native renal tissue. These constructs are derived from epithelial cells of the proximal convoluted tubule, supported by a collagen type IV interface of renal fibroblasts and endothelium (38). By leveraging human pluripotent stem cells (hPSCs) and human induced pluripotent stem cells (hiPSCs) (39), dissociated and re-aggregated embryonic kidneys can be used to develop compact renal organoids that not only contain functional nephrons but follow the course of development of a normal kidney. Despite this, the collecting ducts in these organoids are still absent of a drainage system. Although currently employed to study the effects of drug toxicity, risk assessment, and the development of new drugs (40), the production of renal tissue bioprints demands approaches that cater to both the filtration and homeostatic function of kidneys.
Liver
Despite the inherent and remarkable capability of the liver to regenerate and restore its normal function post hepatic injury, the recuperative process can, on occasion, fail to initiate or becomes dysfunctional, leading to pathogenesis and ultimately hepatic failure (41). Impairment of liver function due to chronic liver disease, alcohol abuse, cancer, and other liver insults stipulates the need for developing liver tissue fabricates that fulfil the physiological and structural demands of the original tissue. Limited progress in bioprinting viable liver tissue has prompted the development of ‘liver-on-a-chip’ and microplatform bioreactors, which offer a favorable microenvironment that simulates in vivo conditions and make microtechnology a promising tool to bioprint tissue substitutes that can mimic the complex functions and architecture of hepatic tissues (42). For example, Organovo has utilized an extrusion-based bioprinting technique to develop a 3D printed hepatic tissue construct that sustained functionality for up to 4 weeks (43). Additionally, endothelial cells have been combined with primary hepatocytes to achieve vascularization (36). Although the printed models did not exhibit accurate structural morphology, the successful use of endothelial cells has given way to the possibility of developing a fully vascularized and functional hepatic model of the liver that can potentially be implanted (36).
Pancreas
The World Health Organization declares that approximately 1.6 million deaths result from diabetes mellitus each year (44). Raised blood glucose levels, which are typically regulated by the pancreas, can cause vascular damage and often result in nephropathy, retinopathy, hypertension, stroke, and neuropathy (45). 3D bioprinting focuses on synthesizing a construct to implant allogeneic islet cells that may better regulate pancreatic function and counteract the debilitating effects caused by diabetes (46). One technique, named extrusion bioprinting, is able to utilize alginate and methylcellulose (Alg/MC) as a bioink to fabricate tissue constructs with accurate morphology, insulin-secreting properties and the ability for insulin to diffuse through the scaffold, however, it also results in decreased viability and fragmentation of the printed islets which eventually undergo apoptosis (47). To address this, co-axial bioprinting technology allows islet cells to be co-printed with endothelial progenitor cells and T cells in order to improve vascularization and viability (46). The bioprinting of a fully functional pancreas is still under investigation and much more research is needed to develop appropriate biomaterials that support and facilitate insulin production. If its potential is realized, 3D printing of viable pancreatic tissue may change the course of treatment for type 1 diabetes mellitus.
Cornea
Because the cornea is an avascular structure, bioprinting techniques are deemed a promising alternative to the complications associated with corneal transplantation. Restoration of vision in cases of corneal blindness is predominantly performed via corneal transplants, yet the ratio of corneal donation to patients in need for such transplants is immensely low (less than 1:70 of cases) (48) and calls for investigating novel treatments to cover the shortage of donors (48). Laser assisted bioprinting to fabricate corneal constructs has been carried out by depositing limbal epithelial stem cells (LESCs) and human adipose tissue-derived stem cells (hAScs) using collagen 1 and recombinant human laminin as a basis for these bioinks. Along with corneal constructs, biofabrication of retinal ganglion cells, glia as well as of retinal pigment epithelium has been described (49). Moreover, 3D bioprinting provides the advantage of customizing corneal implants as well as precise control over structure and refractive ability, which makes the use of such implants in treating corneal blindness of immense importance.
In Clinical and Cancer Research
Tissue bioprints have been used extensively to study the effects of drug toxicity and the pathophysiology of different diseases. There is a pressing demand for establishing human tissue models that can accurately recapitulate the cellular and physiological complexities of in vivo tissues so that the pathogenesis of different disease states can be studied more efficiently; and for drug response and toxicity to be investigated more effectively (50). Restrictions associated with cancer research carried out on 2D cultures and murine models, which include failing to develop tumor microenvironments (TME) and failing to recapitulate human tissue morphology, have been addressed by 3D bioprinted models (51) with the added advantage of permitting the study of personalized cancer treatment (52). Engineered hydrogel tumor models present an opportunity to recapitulate the in vivo environment providing deeper insight into cancer cell behaviour (53), for example, the recently described hyaluronan (HA)-oxime breast cancer model that effectively represents the in vivo phenotype (54).
Bioprinting may also be employed to prepare drugs and drug delivery systems (55). Predicting the efficacy and toxicity of new drugs will hasten the process of introducing new and improved drugs into the market, as biofabricated tissue constructs will not only allow for testing of these drugs on native-like tissues but will also provide an opportunity to study physiological responses to their effects (40). Moreover, patient-specific models are also being utilized to study surgical planning, to rehearse surgical procedures, and for medical and patient education (56).
In situ Bioprinting
The objective of 3D bioprinting is to synthesize viable tissue constructs outside of any living organism that can be successfully implanted within the body (57). in situ bioprinting, also called in vivo bioprinting, allows the printing to take place directly at the site of defect or injury (58) and has been practiced in the context of skin, where inkjet bioprinting technology (59) has been used to bioprint in situ over skin using an inkjet-based bioprinter (60). Although these investigations suggest immense potential for bioprinting tissues on site, further investigation is needed to make it practical for application.
Future Plans and Possibilities
3D bioprinting is anticipated to contribute significantly to the development of personalized drugs, which will be particularly effective in overcoming the issue of pharmacogenetic polymorphisms (61). Ex vivo 3D Bioprinting also provides the opportunity to customize prostheses and dental implants in accordance with the needs of the patient (62). The most anticipated advancement of 3D bioprinting is predicted to be made in the field of tissue engineering and regenerative medicine (TERM), where these printing technologies can be employed to construct viable and functional tissues. However, current research limits the clinical application of synthesized tissue constructs as the significant challenges of vascularization, innervation, in vivo survival, and functional sustainability remain. If biofabrication of complex tissues is to become successful in the future, this would bridge the gap between organ demand and organ donation (63); and better test the efficacy of drugs directly on a patient’s printed tissue strip (62). Although there are many questions that remain unanswered about the clinical application of this technology, one cannot deny the immense potential it holds if a transdisciplinary effort is carried out to circumvent the aforementioned challenges.
Limitations
Major limitations associated with 3D bioprinting of tissues and organs are vascularization and maintenance of post-print sustainability. Bioprinting thick tissues that are viable and functional also remains a colossal challenge (64). Although 3D bioprinting offers a novel approach to conventional treatment, and the media heavily promotes the timely emergence of organ bioprinting, immense work needs to be done for it to become applicable in clinical settings (65). Besides biological concerns, monitored use of these printers and patent concerns also need to be addressed (66). An important question, in this case, is whether bioprinters will be a patentable or a non-patentable technique? The answer to this question remains, along with other legal concerns regarding the printers and the technique itself (67).
Socio-ethical View
As the innovations of 3D bioprinting gain traction, it becomes crucial to highlight ethical and social concerns, and to devise policies regarding the application and distribution of this technology (68). Firstly, the research community is still unable to determine as to if or when organ biofabrication, in its entirety, will be possible. in vivo testing of bioprinted tissues and organs in humans is another area of major concern: there is the question of who will be responsible if printed tissue undergoes necrosis or fails to conform to its bodily environment, ultimately leading to infection at the site of implantation? Would there be a certain limitation as to what should and should not be bioprinted? In the context of complications, who will be held accountable for the treatment opportunity that was lost in favour of implanting biofabricated tissue (69)? Currently, there is an absence of international directives and a regulatory outline related to experimental testing of bioprinted tissue in humans. Concerns over ownership and value of these biofabricated constructs by different parties involved in their development also needs to be addressed (70), as does the financial cost for those who opt for biofabricated and personalized tissues and implants as treatment (71). Taking into account all these concerns, a collective effort of researchers, lawyers and policymakers is needed to avoid exploitation of this potentially revolutionary technology (72).
Conclusion
3D bioprinting opens new doors in the field of regenerative medicine and research in general by providing an opportunity to print viable tissue constructs. Although this technology can imitate the function and architecture of their counterparts in the human body, much work needs to be done to make it clinically applicable and to sustain post-print viability of these tissues, so that they retain functionality and maintain reproducibility. Ethical concerns and patent issues need to be resolved before it can be made available as a treatment plan; and a colossal interdisciplinary collaboration is needed to bring this technique from bench to bedside.
Acknowledgments
We would like to extend our gratitude to Muhammad Romail Manan (Services Institute of Medical Sciences, Lahore) for guiding and supervising us. We would also like to acknowledge our colleagues; Rimsha Ishfaq (Quiad-e-Azam Medical College, Bahawalpur), Aimen Rabbani (Quiad-e-Azam Medical College, Bahawalpur), and Sidra Ashraf (Quiad-e-Azam Medical College, Bahawalpur) for their technical help during the process.
References
- Li N, Qiao D, Zhao S, Lin Q, Zhang B, Xie F. 3D printing to innovate biopolymer materials for demanding applications: A review. Materials Today Chemistry. 2021;20:100459. https://doi.org/10.1016/j.mtchem.2021.100459
- Roseti L, Parisi V, Petretta M, Cavallo C, Desando G, Bartolotti I, et al. Scaffolds for bone tissue engineering: state of the art and new perspectives. Materials Science and Engineering: C. 2017;78:1246-62. https://doi.org/10.1016/j.msec.2017.05.017
- Oklu R, Zhang YS, Yue K, Aleman J, Mollazadeh-Moghaddam K, Bakht SM, et al. 3D Bioprinting for Tissue and Organ Fabrication. 2016. http://hdl.handle.net/1721.1/105262
- Zhang X, Zhang Y. Tissue engineering applications of three-dimensional bioprinting. Cell biochemistry and biophysics. 2015;72(3):777-82. https://doi.org/10.1007/s12013-015-0531-x
- Heinrich MA, Liu W, Jimenez A, Yang J, Akpek A, Liu X, et al. 3D bioprinting: from benches to translational applications. Small. 2019;15(23):1805510. https://doi.org/10.1002/smll.201805510
- Li J, Chen M, Fan X, Zhou H. Recent advances in bioprinting techniques: approaches, applications and future prospects. Journal of translational medicine. 2016;14(1):1-15. https://doi.org/10.1186/s12967-016-1028-0
- Groll J, Burdick JA, Cho D-W, Derby B, Gelinsky M, Heilshorn SC, et al. A definition of bioinks and their distinction from biomaterial inks. Biofabrication. 2018;11(1):013001. https://doi.org/10.1088/1758-5090/aaec52
- Gungor-Ozkerim PS, Inci I, Zhang YS, Khademhosseini A, Dokmeci MR. Bioinks for 3D bioprinting: an overview. Biomaterials science. 2018;6(5):915-46. https://doi.org/10.1039/C7BM00765E
- Ozbolat IT, Hospodiuk M. Current advances and future perspectives in extrusion-based bioprinting. Biomaterials. 2016;76:321-43. https://doi.org/10.1016/j.biomaterials.2015.10.076
- Ozbolat IT, Peng W, Ozbolat V. Application areas of 3D bioprinting. Drug discovery today. 2016;21(8):1257-71. https://doi.org/10.1016/j.drudis.2016.04.006
- Augustine R. Skin bioprinting: a novel approach for creating artificial skin from synthetic and natural building blocks. Progress in biomaterials. 2018;7(2):77-92. https://doi.org/10.1007/s40204-018-0087-0
- He P, Zhao J, Zhang J, Li B, Gou Z, Gou M, et al. Bioprinting of skin constructs for wound healing. Burns & trauma. 2018;6. https://doi.org/10.1186/s41038-017-0104-x
- Xu J, Zheng S, Hu X, Li L, Li W, Parungao R, et al. Advances in the research of bioinks based on natural collagen, polysaccharide and their derivatives for skin 3D bioprinting. Polymers. 2020;12(6):1237. https://doi.org/10.3390/polym12061237
- Liu F, Liu C, Chen Q, Ao Q, Tian X, Fan J, et al. Progress in organ 3D bioprinting. International Journal of Bioprinting. 2018;4(1). 10.18063/IJB.v4i1.128
- Bose S, Vahabzadeh S, Bandyopadhyay A. Bone tissue engineering using 3D printing. Materials today. 2013;16(12):496-504. https://doi.org/10.1016/j.mattod.2013.11.017
- Wang X, Ao Q, Tian X, Fan J, Wei Y, Hou W, et al. 3D bioprinting technologies for hard tissue and organ engineering. Materials. 2016;9(10):802. https://doi.org/10.3390/ma9100802.
- Sopyan I, Mel M, Ramesh S, Khalid K. Porous hydroxyapatite for artificial bone applications. Science and Technology of Advanced Materials. 2007;8(1-2):116. https://doi.org/10.1016/j.stam.2006.11.017
- Freeman FE, Burdis R, Kelly DJ. Printing New Bones: From Print-and-Implant Devices to Bioprinted Bone Organ Precursors. Trends in Molecular Medicine. 2021. https://doi.org/10.1016/j.molmed.2021.05.001
- Leucht A, Volz A-C, Rogal J, Borchers K, Kluger P. Advanced gelatin-based vascularization bioinks for extrusion-based bioprinting of vascularized bone equivalents. Scientific reports. 2020;10(1):1-15. https://doi.org/10.1038/s41598-020-62166-w
- Gao G, Huang Y, Schilling AF, Hubbell K, Cui X. Organ bioprinting: are we there yet? Advanced healthcare materials. 2018;7(1):1701018. https://doi.org/10.1002/adhm.201701018
- You F, Eames BF, Chen X. Application of extrusion-based hydrogel bioprinting for cartilage tissue engineering. International journal of molecular sciences. 2017;18(7):1597. https://doi.org/10.3390/ijms18071597
- Roseti L, Cavallo C, Desando G, Parisi V, Petretta M, Bartolotti I, et al. Three-dimensional bioprinting of cartilage by the use of stem cells: a strategy to improve regeneration. Materials. 2018;11(9):1749. https://doi.org/10.3390/ma11091749
- Daly AC, Critchley SE, Rencsok EM, Kelly DJ. A comparison of different bioinks for 3D bioprinting of fibrocartilage and hyaline cartilage. Biofabrication. 2016;8(4):045002. https://doi.org/10.1088/1758-5090/8/4/045002
- World Health Organization. Cardiovascular diseases (CVDs). World Health Organization; 2021 [updated 2021 Jun 11; cited 2021 July 11]. Available from: https://www.who.int/en/news-room/fact-sheets/detail/cardiovascular-diseases-(cvds)
- Liaw NY, Zimmermann W-H. Mechanical stimulation in the engineering of heart muscle. Advanced drug delivery reviews. 2016;96:156-60. https://doi.org/10.1016/j.addr.2015.09.001
- Radisic M, Park H, Shing H, Consi T, Schoen FJ, Langer R, et al. Functional assembly of engineered myocardium by electrical stimulation of cardiac myocytes cultured on scaffolds. Proceedings of the National Academy of Sciences. 2004;101(52):18129-34. https://doi.org/10.1073/pnas.0407817101
- Duan B, Kapetanovic E, Hockaday LA, Butcher JT. Three-dimensional printed trileaflet valve conduits using biological hydrogels and human valve interstitial cells. Acta biomaterialia. 2014;10(5):1836-46. https://doi.org/10.1016/j.actbio.2013.12.005
- Pasca AM, Sloan SA, Clarke LE, Tian Y, Makinson CD, Huber N, et al. Functional cortical neurons and astrocytes from human pluripotent stem cells in 3D culture. Nature methods. 2015;12(7):671-8. https://doi.org/10.1038/nmeth.3415
- Gu Q, Tomaskovic-Crook E, Lozano R, Chen Y, Kapsa RM, Zhou Q, et al. Functional 3D neural mini-tissues from printed gel-based bioink and human neural stem cells. Advanced healthcare materials. 2016;5(12):1429-38. https://doi.org/10.1002/adhm.201600095
- Wang S, Guan S, Li W, Ge D, Xu J, Sun C, et al. 3D culture of neural stem cells within conductive PEDOT layer-assembled chitosan/gelatin scaffolds for neural tissue engineering. Materials Science and Engineering: C. 2018;93:890-901. https://doi.org/10.1016/j.msec.2018.08.054
- Galliger Z, Vogt CD, Panoskaltsis-Mortari A. 3D bioprinting for lungs and hollow organs. Translational Research. 2019;211:19-34. https://doi.org/10.1016/j.trsl.2019.05.001
- Horváth L, Umehara Y, Jud C, Blank F, Petri-Fink A, Rothen-Rutishauser B. Engineering an in vitro air-blood barrier by 3D bioprinting. Scientific reports. 2015;5(1):1-8. https://doi.org/10.1038/srep07974
- Mahfouzi SH, Tali SHS, Amoabediny G. 3D bioprinting for lung and tracheal tissue engineering: Criteria, advances, challenges, and future directions. Bioprinting. 2021;21:e00124. https://doi.org/10.1016/j.bprint.2020.e00124
- Hoch E, Tovar GE, Borchers K. Bioprinting of artificial blood vessels: current approaches towards a demanding goal. European Journal of Cardio-Thoracic Surgery. 2014;46(5):767-78 https://doi.org/10.1093/ejcts/ezu242
- Aljohani W, Ullah MW, Zhang X, Yang G. Bioprinting and its applications in tissue engineering and regenerative medicine. International journal of biological macromolecules. 2018;107:261-75. https://doi.org/10.1016/j.ijbiomac.2017.08.171
- Mota C, Camarero-Espinosa S, Baker MB, Wieringa P, Moroni L. Bioprinting: from tissue and organ development to in vitro models. Chemical reviews. 2020;120(19):10547-607. https://doi.org/10.1021/acs.chemrev.9b00789
- Chang TMS. ARTIFICIAL CELL evolves into nanomedicine, biotherapeutics, blood substitutes, drug delivery, enzyme/gene therapy, cancer therapy, cell/stem cell therapy, nanoparticles, liposomes, bioencapsulation, replicating synthetic cells, cell encapsulation/scaffold, biosorbent/immunosorbent haemoperfusion/plasmapheresis, regenerative medicine, encapsulated microbe, nanobiotechnology, nanotechnology. Artificial cells, nanomedicine, and biotechnology. 2019;47(1):997-1013. https://doi.org/10.1080/21691401.2019.1577885
- Xia Z, Jin S, Ye K. Tissue and organ 3D bioprinting. SLAS TECHNOLOGY: Translating Life Sciences Innovation. 2018;23(4):301-14. https://doi.org/10.1177/2472630318760515
- Turunen S, Kaisto S, Skovorodkin I, Mironov V, Kalpio T, Vainio S, et al. 3D bioprinting of the kidney—hype or hope? 2018. https://urn.fi/URN:NBN:fi:tty-201901211125
- Peng W, Unutmaz D, Ozbolat IT. Bioprinting towards physiologically relevant tissue models for pharmaceutics. Trends in biotechnology. 2016;34(9):722-32. https://doi.org/10.1016/j.tibtech.2016.05.013
- Diehl AM. Liver regeneration. Front Biosci. 2002;7:e301-14. Epub 2002/06/28. doi: 10.2741/a925. PubMed PMID: 12086922. https://pubmed.ncbi.nlm.nih.gov/12086922/
- Lee K-H, Lee J, Lee S-H. 3D liver models on a microplatform: well-defined culture, engineering of liver tissue and liver-on-a-chip. Lab on a Chip. 2015;15(19):3822-37. https://doi.org/10.1039/C5LC00611B
- Kryou C, Leva V, Chatzipetrou M, Zergioti I. Bioprinting for liver transplantation. Bioengineering. 2019;6(4):95. https://doi.org/10.3390/bioengineering6040095
- World Health Organization. Diabetes. World Health Organization; 2021 [updated 2021 Nov 10; cited 2021 July 11]. Available from: https://www.who.int/news-room/fact-sheets/detail/diabetes
- Dal Canto E, Ceriello A, Rydén L, Ferrini M, Hansen TB, Schnell O, Standl E, Beulens JW. Diabetes as a cardiovascular risk factor: an overview of global trends of macro and micro vascular complications. European journal of preventive cardiology. 2019;26(2_suppl):25-32. https://doi.org/10.1177/2047487319878371
- Kim J, Kang K, Drogemuller CJ, Wallace GG, Coates PT. Bioprinting an artificial pancreas for type 1 diabetes. Current diabetes reports. 2019;19(8):1-10. https://doi.org/10.1007/s11892-019-1166-x
- Duin S, Schütz K, Ahlfeld T, Lehmann S, Lode A, Ludwig B, et al. 3D Bioprinting of functional islets of langerhans in an alginate/methylcellulose hydrogel blend. Advanced healthcare materials. 2019;8(7):1801631. https://doi.org/10.1002/adhm.201801631
- Zhang B, Xue Q, Li J, Ma L, Yao Y, Ye H, et al. 3D bioprinting for artificial cornea: Challenges and perspectives. Medical engineering & physics. 2019;71:68-78. https://doi.org/10.1016/j.medengphy.2019.05.002
- Sorkio A, Koch L, Koivusalo L, Deiwick A, Miettinen S, Chichkov B, et al. Human stem cell based corneal tissue mimicking structures using laser-assisted 3D bioprinting and functional bioinks. Biomaterials. 2018;171:57-71. https://doi.org/10.1016/j.biomaterials.2018.04.034
- Memic A, Navaei A, Mirani B, Cordova JAV, Aldhahri M, Dolatshahi-Pirouz A, et al. Bioprinting technologies for disease modeling. Biotechnology letters. 2017;39(9):1279-90. https://doi.org/10.1007/s10529-017-2360-z
- Sánchez-Salazar MG, Álvarez MM, Trujillo-de Santiago G. Advances in 3D bioprinting for the biofabrication of tumor models. Bioprinting. 2021;21:e00120. https://doi.org/10.1016/j.bprint.2020.e00120
- Mao S, Pang Y, Liu T, Shao Y, He J, Yang H, et al. Bioprinting of in vitro tumor models for personalized cancer treatment: a review. Biofabrication. 2020;12(4):042001. https://doi.org/10.1088/1758-5090/ab97c0
- Bahlmann LC, Smith LJ, Shoichet MS. Designer biomaterials to model cancer cell invasion in vitro: predictive tools or just pretty pictures? Advanced Functional Materials. 2020;30(16):1909032. https://doi.org/10.1002/adfm.201909032
- Baker AE, Bahlmann LC, Tam RY, Liu JC, Ganesh AN, Mitrousis N, et al. Benchmarking to the gold standard: hyaluronan-oxime hydrogels recapitulate xenograft models with in vitro breast cancer spheroid culture. Advanced Materials. 2019;31(36):1901166. https://doi.org/10.1002/adma.201901166
- Dundar M, Prakash S, Lal R, Martin DK. Future biotechnology. The EuroBiotech Journal. 2019;3(2):53-6.
- Wake N, Rosenkrantz AB, Huang R, Park KU, Wysock JS, Taneja SS, et al. Patient-specific 3D printed and augmented reality kidney and prostate cancer models: impact on patient education. 3D printing in medicine. 2019;5(1):1-8. https://doi.org/10.1186/s41205-019-0041-3
- Ashammakhi N, Ahadian S, Pountos I, Hu S-K, Tellisi N, Bandaru P, et al. In situ three-dimensional printing for reparative and regenerative therapy. Biomedical microdevices. 2019(2):42. https://doi.org/10.1007/s10544-019-0372-2
- Di Bella C, Duchi S, O'Connell CD, Blanchard R, Augustine C, Yue Z, et al. In situ handheld three-dimensional bioprinting for cartilage regeneration. Journal of Tissue Engineering and Regenerative Medicine. 2018;12(3):611-21. https://doi.org/10.1002/term.2476
- Singh S, Choudhury D, Yu F, Mironov V, Naing MW. In situ bioprinting–bioprinting from benchside to bedside? Acta biomaterialia. 2020;101:14-25. https://doi.org/10.1016/j.actbio.2019.08.045
- Wang M, He J, Liu Y, Li M, Li D, Jin Z. The trend towards in vivo bioprinting. International Journal of Bioprinting. 2015;1(1). http://dx.doi.org/10.18063/IJB.2015.01.001.
- Ursan ID, Chiu L, Pierce A. Three-dimensional drug printing: a structured review. Journal of the American Pharmacists Association. 2013;53(2):136-44. https://doi.org/10.1331/JAPhA.2013.12217
- Schubert C, Van Langeveld MC, Donoso LA. Innovations in 3D printing: a 3D overview from optics to organs. British Journal of Ophthalmology. 2014;98(2):159-61. http://dx.doi.org/10.1136/bjophthalmol-2013-304446
- Ozbolat IT, Yu Y. Bioprinting toward organ fabrication: challenges and future trends. IEEE Transactions on Biomedical Engineering. 2013;60(3):691-9. 10.1109/TBME.2013.2243912
- Moldovan F. Recent trends in bioprinting. Procedia Manufacturing. 2019;32:95-101. https://doi.org/10.1016/j.promfg.2019.02.188
- Banks J. Adding value in additive manufacturing: researchers in the United Kingdom and Europe look to 3D printing for customization. IEEE pulse. 2013;4(6):22-6. 10.1109/MPUL.2013.2279617
- Hoy MB. 3D printing: making things at the library. Medical reference services quarterly. 2013;32(1):93-9. https://doi.org/10.1080/02763869.2013.749139
- Li PH. 3D bioprinting technologies: patents, innovation and access. Law, Innovation and Technology. 2014;6(2):282-304. https://doi.org/10.5235/17579961.6.2.282
- Vijayavenkataraman S, Lu W, Fuh JYH. 3D bioprinting–an ethical, legal and social aspects (ELSA) framework. Bioprinting. 2016;1:11-21. https://doi.org/10.1016/j.bprint.2016.08.001
- Gilbert F, O’Connell CD, Mladenovska T, Dodds S. Print me an organ? Ethical and regulatory issues emerging from 3D bioprinting in medicine. Science and engineering ethics. 2018;24(1):73-91. https://doi.org/10.1007/s11948-017-9874-6
- Harbaugh JT. Do you own your 3D bioprinted body?: analyzing property issues at the intersection of digital information and biology. American journal of law & medicine. 2015;41(1):167-89. https://doi.org/10.1177/0098858815591512
- Vermeulen N, Haddow G, Seymour T, Faulkner-Jones A, Shu W. 3D bioprint me: a socioethical view of bioprinting human organs and tissues. Journal of Medical Ethics. 2017;43(9):618-24. http://dx.doi.org/10.1136/medethics-2015-103347
- Tran JL. To bioprint or not to bioprint. NCJL & Tech. 2015;17:123.

This work is licensed under a Creative Commons Attribution-NonCommercial-ShareAlike 4.0 International License.